Figure 2 shows a schematic picture of the discharge process. The current enters the cell from the current feeder at the negative electrode. The charge transfer reaction occurs at the interface between the electrode material and electrolyte contained in the porous electrode, also called the pore electrolyte. Here, an oxidation of the electrode material may take place through an anodic charge transfer reaction, denoted i
loc, a in
Figure 2. The shapes of the two curves in the graph are described by the electrode kinetics for the specific materials. The reaction may also involve the transport of chemical species from the pore electrolyte and also from the electrode particles.
The graph in Figure 3 plots the charge transfer current density,
iloc, as a function of the electrode potential,
E. These curves describe the polarization of the electrodes during discharge.
The negative electrode is polarized anodically during discharge, a positive current as indicated by the arrow in Figure 3. The potential of the negative electrode increases. The positive electrode is polarized cathodically, a negative current as indicated by the arrow. The potential of the positive electrode decreases.
Consequently, Figure 3 also shows that the potential difference between the electrodes, here denoted
Ecell, decreases during discharge compared to the open cell voltage, here denoted
Eocv. The value of
Ecell is the cell voltage at a given current
iloc, if the ohmic losses in the cell are negligible. This is usually not the case in most batteries. This implies that the cell voltage in most cases is slightly smaller than that shown in
Figure 3.
During charge, the processes are reversed; see Figure 4. Electrical energy is transformed to chemical energy that is stored in the battery.
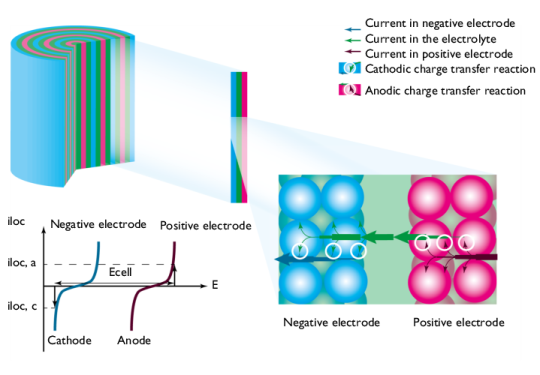
The difference in potential between the electrodes, here denoted Ecell, at a given i
loc, increases during charge, compared to the open cell voltage, here denoted
Eocv; see
Figure 5. The value of
Ecell is equal to the cell voltage when ohmic losses are neglected. In most cells, these losses are not negligible and they would add to the cell voltage.